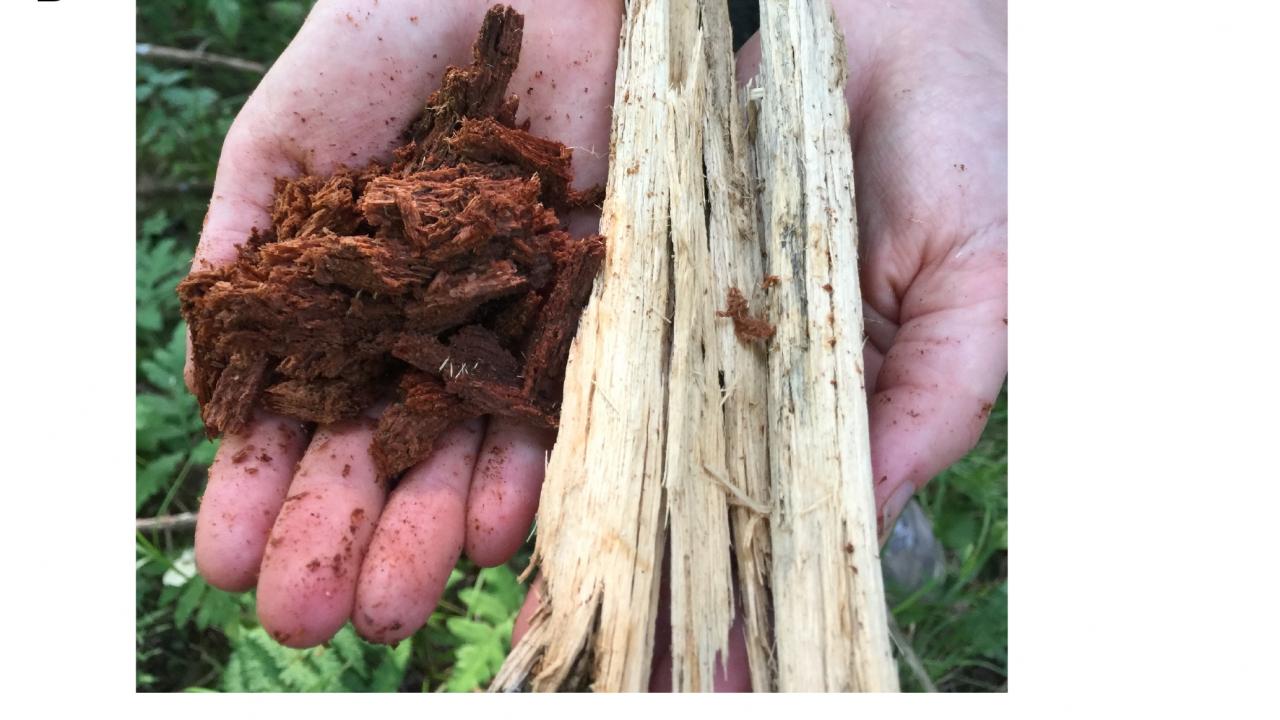
Engineering Fungi as Enzymatic Powerhouses for a Plant-Based Economy
The public perception of fungi is typically focused on the beautiful, and occasionally delicious, fruiting bodies of a relatively limited range of fungal species. But the human applications of fungi and the compounds they produce go well beyond our dinner plate or photo album. Fungi, many of which gain energy from decaying plant matter, are known to produce a wide suite of enzymes specializing in the degradation of biomass components. Of particular interest is their capacity to break down lignocellulose, a complex and abundant constituent of plant tissue that forms secondary cell walls.
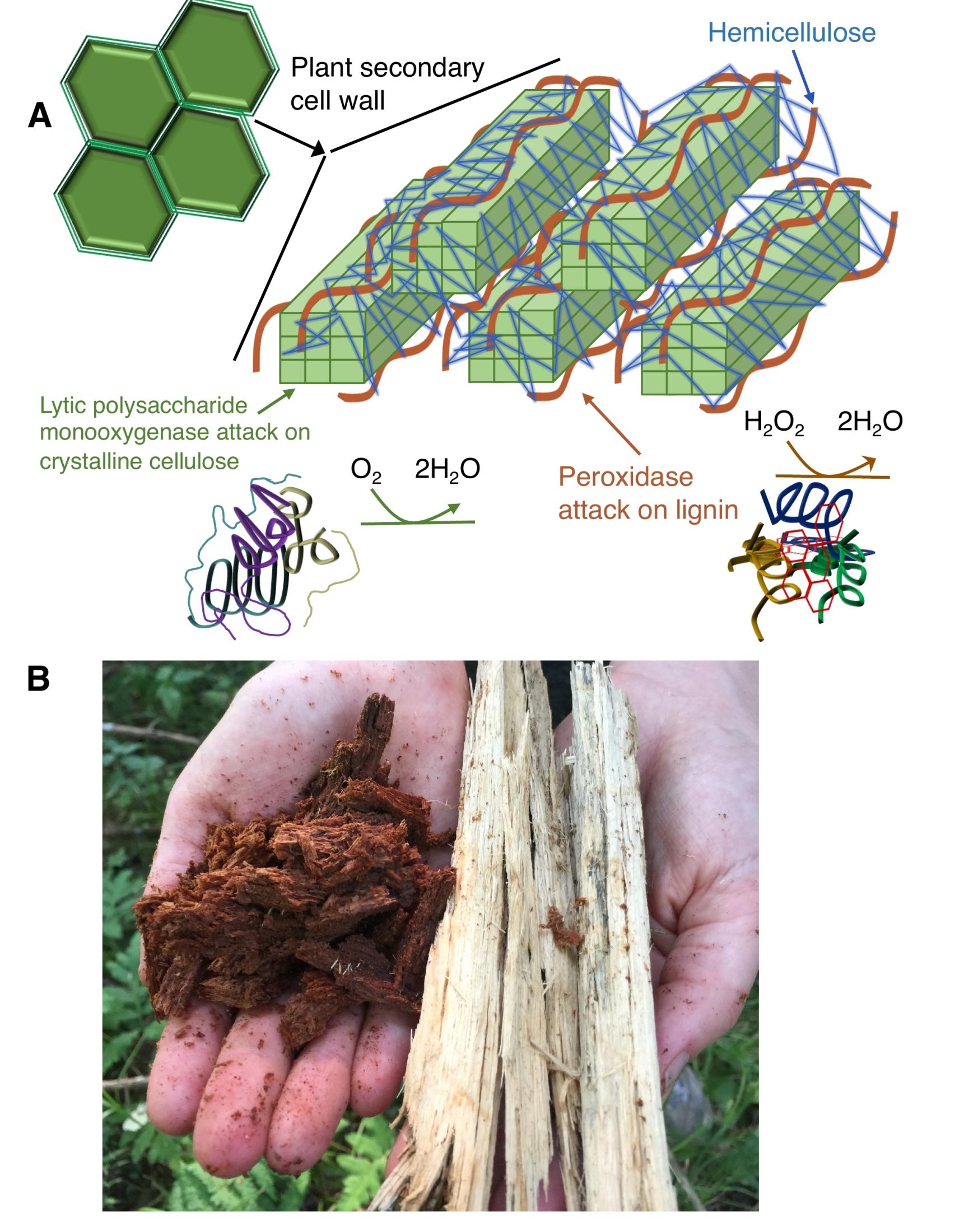
The most abundant biomass material on earth, lignocellulose is a complex structural matrix composed of cellulose, hemicellulose, and lignin, and gives plants their rigidity (Figure 1). Cellulose has a regular, semi-crystalline structure, which many environmental microbes- including bacteria and archaea- can depolymerize, at least in part. However, some filamentous fungi produce a specialized family of enzymes called auxiliary activity lytic polysaccharide monooxygensases, which oxidatively degrade the highly crystalline portion of cellulose. Furthermore, isotopic labeling has suggested that fungi play a substantial role in overall environmental cellulose decomposition compared to bacteria. Due to its irregular structure, lignin degradation is more biochemically challenging, and only a limited range of fungi- collectively called the white-rot fungi- can effectively delignify plant biomass through production of oxidative enzymes such as peroxidases and phenol oxidases (Figure 1). Brown-rot fungi are a group of Basidiomycetes that can also partially modify lignin. Collectively, this diversity of functions makes fungal-derived enzymes crucial in producing products such as paper, animal feeds, human consumables like flour and wine, detergent, and biofuels. In fact, fungi account as the source of more than 50% of commercially-produced enzymes for biotechnological applications.
Although many fungal species are equipped with an impressive range of these so-called ‘lignocellulolytic enzymes’ (LCEs), expression in wild-type strains is often highly regulated by carbon catabolite repressors. Common mechanisms including zinc-finger transcription factors function to downregulate production of LCEs as long as simple sugars are available for metabolism. Conversely, upregulation is triggered by starvation conditions. To meet the demands of commercial industrial scaling, a variety of bio-engineering techniques have been employed to improve LCE production.
A long-used strain development technique has been mutagenesis through use of UV irradiation, or a chemical agent such as ethidium bromide. Such methods have successfully produced ‘hypercellulolytic’ strains, especially within the commercially important genus Aspergillus. Although mutagenesis is an attractive technique due to its relative simplicity and cost-efficiency, a major downside is that producing only positive mutation(s) is unlikely; large improvements in enzymatic production may be accompanied by detrimental characteristics such as decreased rate of growth. Another fairly straightforward engineering technique that has been employed is adaptive evolution. For example, repeated culturing using a C-based compound of interest (such as cellulose) as a sole C source selects for strains with elevated cellobiohydrolase activity.
Explicit, targeted genetic modification through a variety of techniques has aided in the improvement of several aspects of LCE-producing fungal strains including increased expression, range of activity, or efficiency of LCEs. This may be mechanistically achieved through alteration or disruption of promoters and regulators which control expression, or increased gene copy number and/or integration of new genes. However, the ladder strategy is hampered by the dependency of fungal genomic integration on non-homologous end joining (NHEJ), which limits recombination at a precise locus. A technological workaround to this barrier has been either the interruption or transient silencing of protein kinases essential to NHEJ, in turn allowing homologous recombination to be induced. Enzymatic production may also be improved in strains capable of efficient homologous or heterologous expression, such as Aspergillus niger and Penicillium verruculosum.
The CRISPR/Cas9 genome editing technique has also recently been applied to fungal bioengineering. This technique, originally identified as part of prokaryotic immune response, utilizes a designed ‘guide RNA’ to direct the Cas9 protein to make precise cuts in a gene sequence. Although most of the work published on filamentous fungi thus far has been to establish the CRISPR/Cas9 genome editing system in various species of interest, some headway has been made in strain development. For example, it has been used to induce hyper-production (~13x increase above wild type strain) of cellulase by deletion of repressor genes in the species Myceliopthora thermophila. This species also produces lignocellulolytic enzymes with adequate thermostability, and therefore may be a promising organism for future engineering efforts. Progress has also been made optimizing the editing system in commercially important strains such as Aspergillus oryzae, which is used for the fermentation of plant-based products like sake and soy sauce.
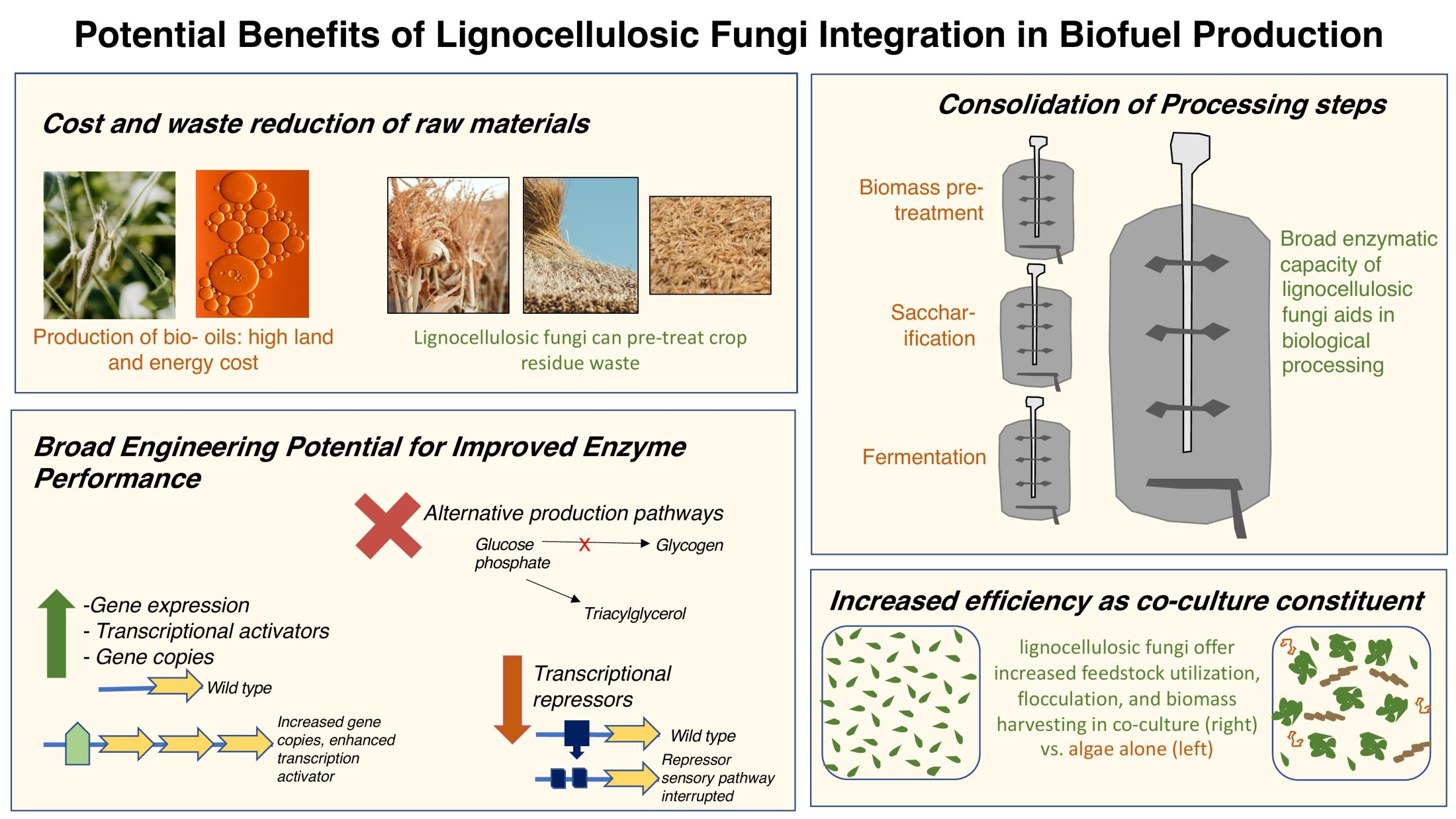
Moving towards an economy of renewable, plant-based sources of energy and polymers requires precise and efficient methods of extraction, conversion, and separation. Biofuels are an example of a rapidly growing and globally imperative industry with a critical need for increased efficiency in lignocellulose degradation (Figure 2). This is because a key aspect of production is the initial breakdown of polysaccharides in plant cell walls before the resulting sugars can be fermented. However, current enzymatic methods of achieving this initial step are not cost-effective. Critical achievements on this front include production enhancement, improved activity and stability of lignocellulosic enzymes (LCEs), and discovery of commercially-viable, previously unknown ‘extremozymes’.
An alternative approach to the biofuel production process just outlined is the generation of ‘microdiesel’ with oleaginous microorganisms that can directly metabolize lignocellulose for lipid production. This step is followed by a chemical transesterfication and refining processes to produce the final product. Although a diverse range of fungi are capable of lipid accumulation from lignocellulose feedstock, they often have slow growth rates. Studies have shown success with co-culturing approaches to overcome this issue. For example, in a process called simultaneous saccharification and fermentation, the lignocellulolytic and oleaginous fungal species Aspergillus tubingensis was grown with three oleaginous yeasts to convert ‘palm empty fruit bunch’ (waste from palm oil production) to a lipid-based product suitable for biodiesel conversion. The use of specialized fungi to convert agricultural by-products to useable energy sources on an industrial scale is an exciting prospect for the future fuel economy.
Biotechnological advancements are rapidly increasing the potential for utilizing microorganisms such as fungi to achieve a greener, more renewable future. Further, environmental ‘omics (the DNA, RNA, protein, and metabolite-based profiles of environmental microbes) will aide in identification of candidates for engineering, and a mechanistic understanding of gene diversity and function in these organisms.
References
Biodiesel Production and Distribution. Vol. 2021. Alternative Fuels Data Center, US Department of Energy, Washington, D.C.
Abdel-Hamid, A. M., Solbiati, J. O., and Cann, I. K. (2013). Insights into lignin degradation and its potential industrial applications. Adv Appl Microbiol 82, 1-28.
Bhatia, S. K., Bhatia, R. K., and Yang, Y.-H. (2017). An overview of microdiesel — A sustainable future source of renewable energy. Renewable and Sustainable Energy Reviews 79, 1078-1090.
Bulakhov, A. G., Volkov, P. V., Rozhkova, A. M., Gusakov, A. V., Nemashkalov, V. A., Satrutdinov, A. D., and Sinitsyn, A. P. (2017). Using an Inducible Promoter of a Gene Encoding Penicillium verruculosum Glucoamylase for Production of Enzyme Preparations with Enhanced Cellulase Performance. PLoS One 12, e0170404.
Chen, L., Wei, Y., Shi, M., Li, Z., and Zhang, S. H. (2019). An Archaeal Chitinase With a Secondary Capacity for Catalyzing Cellulose and Its Biotechnological Applications in Shell and Straw Degradation. Front Microbiol 10, 1253.
Chum, P. Y., Schmidt, G., Saloheimo, M., and Landowski, C. P. (2017). Transient silencing of DNA repair genes improves targeted gene integration in the filamentous fungus Trichoderma reesei. Applied and environmental microbiology 83, e00535-17.
de Vries, R. P., Patyshakuliyeva, A., Garrigues, S., and Agarwal-Jans, S. (2020). The current biotechnological status and potential of plant and algal biomass degrading/modifying enzymes from Ascomycete Fungi. In "Grand Challenges in Fungal Biotechnology", pp. 81-120. Springer.
Guerriero, G., Hausman, J. F., Strauss, J., Ertan, H., and Siddiqui, K. S. (2015). Destructuring plant biomass: focus on fungal and extremophilic cell wall hydrolases. Plant Sci 234, 180-93.
Intasit, R., Cheirsilp, B., Louhasakul, Y., and Boonsawang, P. (2020). Consolidated bioprocesses for efficient bioconversion of palm biomass wastes into biodiesel feedstocks by oleaginous fungi and yeasts. Bioresour Technol 315, 123893.
Jinek, M., Chylinski, K., Fonfara, I., Hauer, M., Doudna, J. A., and Charpentier, E. (2012). A programmable dual-RNA–guided DNA endonuclease in adaptive bacterial immunity. science 337, 816-821.
Katayama, T., Tanaka, Y., Okabe, T., Nakamura, H., Fujii, W., Kitamoto, K., and Maruyama, J.-i. (2016). Development of a genome editing technique using the CRISPR/Cas9 system in the industrial filamentous fungus Aspergillus oryzae. Biotechnology letters 38, 637-642.
Koechli, C., Campbell, A. N., Pepe-Ranney, C., and Buckley, D. H. (2019). Assessing fungal contributions to cellulose degradation in soil by using high-throughput stable isotope probing. Soil Biology and Biochemistry 130, 150-158.
Kuck, U., and Hoff, B. (2010). New tools for the genetic manipulation of filamentous fungi. Appl Microbiol Biotechnol 86, 51-62.
Kujoth, G. C., Sullivan, T. D., Merkhofer, R., Lee, T.-J., Wang, H., Brandhorst, T., Wüthrich, M., and Klein, B. S. (2018). CRISPR/Cas9-mediated gene disruption reveals the importance of zinc metabolism for fitness of the dimorphic fungal pathogen Blastomyces dermatitidis. MBio 9, e00412-18.
Kun, R. S., Gomes, A. C. S., Hilden, K. S., Salazar Cerezo, S., Makela, M. R., and de Vries, R. P. (2019). Developments and opportunities in fungal strain engineering for the production of novel enzymes and enzyme cocktails for plant biomass degradation. Biotechnol Adv 37, 107361.
Liu, Q., Gao, R., Li, J., Lin, L., Zhao, J., Sun, W., and Tian, C. (2017). Development of a genome-editing CRISPR/Cas9 system in thermophilic fungal Myceliophthora species and its application to hyper-cellulase production strain engineering. Biotechnology for biofuels 10, 1-14.
Maheshwari, R., Bharadwaj, G., and Bhat, M. K. (2000). Thermophilic fungi: their physiology and enzymes. Microbiology and molecular biology reviews 64, 461-488.
Monrroy, M., Ortega, I., Ramirez, M., Baeza, J., and Freer, J. (2011). Structural change in wood by brown rot fungi and effect on enzymatic hydrolysis. Enzyme Microb Technol 49, 472-7.
Morgenstern, I., Powlowski, J., and Tsang, A. (2014). Fungal cellulose degradation by oxidative enzymes: from dysfunctional GH61 family to powerful lytic polysaccharide monooxygenase family. Brief Funct Genomics 13, 471-81.
Patyshakuliyeva, A., Arentshorst, M., Allijn, I. E., Ram, A. F., de Vries, R. P., and Gelber, I. B. (2016). Improving cellulase production by Aspergillus niger using adaptive evolution. Biotechnol Lett 38, 969-74.
Tran, T. N. T., Breuer, R. J., Avanasi Narasimhan, R., Parreiras, L. S., Zhang, Y., Sato, T. K., and Durrett, T. P. (2017). Metabolic engineering of Saccharomyces cerevisiae to produce a reduced viscosity oil from lignocellulose. Biotechnol Biofuels 10, 69.
Vu, V. H., Pham, T. A., and Kim, K. (2009). Fungal strain improvement for cellulase production using repeated and sequential mutagenesis. Mycobiology 37, 267-71.
Yao, G., Li, Z., Gao, L., Wu, R., Kan, Q., Liu, G., and Qu, Y. (2015). Redesigning the regulatory pathway to enhance cellulase production in Penicillium oxalicum. Biotechnol Biofuels 8, 71.
Zhang, R., Liu, Y., Zhang, Y., Feng, D., Hou, S., Guo, W., Niu, K., Jiang, Y., Han, L., Sindhu, L., and Fang, X. (2019). Identification of a thermostable fungal lytic polysaccharide monooxygenase and evaluation of its effect on lignocellulosic degradation. Appl Microbiol Biotechnol 103, 5739-5750.
Zou, G., Shi, S., Jiang, Y., van den Brink, J., de Vries, R. P., Chen, L., Zhang, J., Ma, L., Wang, C., and Zhou, Z. (2012). Construction of a cellulase hyper-expression system in Trichoderma reesei by promoter and enzyme engineering. Microbial Cell Factories 11, 1-12.